Charging Secondary Batteries
Part of Chapter Battery Systems
WE CHARGE (OR recharge) secondary batteries by applying direct current to the terminals, in the opposite direction from discharge. This is probably not news. The charging process needs to be carefully controlled to avoid undercharge or overcharge, either of which could damage a battery in the long term. We also need to limit the starting current for a recharge to a level that is safe for the battery and the charging equipment, but that minimizes the charging time. Sounds like we’re looking for a compromise.
When we discharge a battery, we’re taking out charge – expressed in ampere hours. Fully charging the battery requires that we replace the ampere hours, but because the charging process isn’t 100% efficient, we need to put in a little more. Generally, lead-acid batteries require about 110% of the ampere hours removed, and NiCd or NiMH batteries require 120% to 130%.
Charging methods can be constant current or constant voltage. Constant current charging is the fastest way to return ampere hours to the battery and is usually used for motive power batteries. Once the battery is charged to a specified voltage (about 2.3 VPC for lead-acid), the charging current is reduced to a finishing rate for a specified time, or the charger switches to constant voltage for a maintenance charge. Or both.
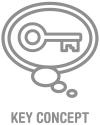
Stationary batteries cannot use constant current because there are upper voltage limits to connected equipment. Constant voltage charging is used, with the charger sized to deliver charging current of about 10% to 20% of the battery Ah rating, while still providing power for the load on the dc bus. Two voltage levels are generally used: float, for long-term operation, and a higher voltage for equalizing cells when necessary.
In either case, charging must be carefully controlled near the finish to minimize potential damage from excessive gassing, while ensuring that the battery reaches full charge. Voltage settings must also account for operating temperatures above or below room temperature.
Read on for more details. Except where noted, the material below pertains to lead-acid batteries.
THE AMPERE-HOUR LAW
1.5.1
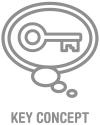
In 1925, George Wood Vinal described the ampere-hour law for lead-acid batteries (actually articulated by J. L. Woodbridge in earlier research). It states, in essence, that a lead-acid cell will safely accept a charge current (at any arbitrary voltage) that is equal to the ampere hours of capacity remaining to be charged. By “safely,” we mean that there will be no excessive gassing or temperature rise in the cell. For example, a battery that has been discharged by 200 Ah can accept an initial charge current of 200 A, without danger of excessive gassing or temperature rise.
The implication of this law is that a completely discharged cell will accept a large charge current, but that the charge current must be progressively reduced to meet the “Ah remaining” conditions to avoid excessive gassing. This is an empirical law, based on experiments performed as early as the 1920s, when all lead-acid batteries were of flooded construction and some gassing was an expected part of the charging process. But the requirement for continuously decreasing current makes constant current charging for modern (especially VRLA) lead-acid batteries impractical, and possibly damaging. Fortunately, constant-voltage charging (properly done) can eliminate the risk of excessive gassing, and temperature-compensated charging reduces the risk of excessive temperature rise.
There are, of course, practical limits on charge current, due to the finite capabilities of internal battery connections, charger components, and the ac power grid. Also, it’s important to understand what we mean by “fully discharged” and “fully charged.” Check the boxout What ‘‘Fully’’ Means for those definitions.
WHAT “FULLY” MEANS
When a discharge cycle is finished, for any reason, you can (almost) always take more out of the battery. But should you?
For our analysis here, we consider a lead-acid battery to be fully discharged when any further discharge would violate any of the limiting conditions that we’ve defined, such as the EOD voltage or the discharge specific gravity.
Defining fully charged is trickier. Strictly speaking, full charge is achieved when the specific gravity of the electrolyte is restored to the manufacturer’s initial specification. But the full-charge specific gravity decreases as the battery ages, and active material is lost to sulfation and corrosion.
From the user’s point of view, full charge could be defined as when the battery can repeat the previous discharge. This requires replacing the Ah discharged with about 110% of that value for a lead-acid battery.
CHARGE ACCEPTANCE
1.5.2
What is charge?
1.5.2.1
We know from the ampere-hour law that a discharged battery will accept charge very rapidly at the start of the charging cycle. But what do we mean by charge?
Unless you’re Reddy Kilowatt®, you can’t hold electricity in your hand. But imagine that you could: cup your hand and imagine a puddle of electricity in it. Now tilt your hand, and the electricity will start to pour out in a thin stream. That stream is discharge current. As it streams out, it reduces the amount of electricity remaining in that puddle in your hand. That puddle is charge.
If you could reverse gravity, you could also get that stream to reverse, and trickle back up into your hand, increasing the amount of electricity (charge) in the puddle. Now the upward stream is charging current, instead of discharging current.
You can see that the longer you let the stream run, the more charge will run out of (or into) your hand. Eventually, your hand will be empty, or full. Likewise, if you tilt your hand a little more, and the volume of the stream increases, your hand will empty sooner. The charge in your hand is limited by how much electricity you could hold at one time.
Now suppose you’re using your anti-gravity tool and charging current is streaming up into your hand. Your hand starts to get full, and some of the current starts to leak out at the edges; you’d like to hold it all, but some escapes anyway. Once your hand has all the charge it can hold, the rest just leaks out.
Sounds silly, right? But it’s essentially what happens in the battery as you reach full charge. The charging current won’t actually “leak out,” but it doesn’t add to the total charge in the battery, which means it must have some other effect.
What happens to the charge that isn’t accepted?
1.5.2.2
First, what happens to the charge that is accepted? At the start of charging, virtually all the current is used to convert the discharged active material back into its charged state. That is, lead sulfate in the negative plate is converted back into lead, and lead sulfate in the positive plate is converted back into lead peroxide. [If you like chemistry, you can check the equations in SECTION 1.2.2, Lead-Acid Batteries.]
You can see that the longer you pump charging current into the battery, the greater the charge will be. In geek talk, charge is the time integral of current. Just so you know, one ampere flowing for one second will give you one coulomb of charge, and an ampere flowing for one hour gives you, of course, one ampere hour. So you can see that one Ah is equal to 3,600 coulombs. See the boxout Power & Energy in a Lead-Acid Cell to learn how much power is expended in charging a 2V battery with one Ah.
POWER & ENERGY IN A LEAD-ACID CELL
In dc circuits, power = voltage x current. Power is expressed in watts, which equals volts x amps.
Therefore, if we charge a 2 volt battery with 1 Ah of charge, we have:
2 volts x 1Ah = (2 volts x 1 amp) x 1 hour = 2 watts x 1 hour = 2 watthours, or 7200 watt-seconds.
Since a watt-second is a joule, the unit of energy:
2 volts x 1Ah = 7200 joules.
From this example, we see that Energy = Power x Time; in geek speak, energy is the time integral of power.
But at about 80% state of charge (SOC), charge acceptance drops below 100%. So once we’ve put back about 80% of the ampere hours that we took out during the previous discharge, the charging action isn’t 100% efficient, and we need to put back a little more than 100% to reach full charge. We normally use a factor of 1.10 for lead-acid batteries. This means that to recharge 100 Ah, we need to put in 110 Ah. The inefficiency of the charging process in this region means that the excess energy is converted to heat.
Electrolysis and Hydrogen Evolution
1.5.2.3
At 80% SOC, we’re also bumping up against other constraints. Most of the active material has already been converted, and the remaining material isn’t as accessible to the electrolyte and the charging current as the first 80%. It’s more difficult for the charging current to reach the active material and electrolyte deep in the plate’s pores. Some of the energy, then, starts to overcharge the already charged material, leading to electrolysis. This doesn’t add to the charge in the battery; instead, it causes gassing, water loss, increased maintenance requirements, grid corrosion, and active material loss in the positive plates. Charging wears out a battery and shortens its life.
And yet, some gassing is an inevitable consequence of fully charging a battery, and in a flooded cell design, has the benefit of mixing the electrolyte, reducing stratification (SECTION 1.5.2.5), and homogenizing the specific gravity of the electrolyte.
Battery Room Ventilation 1.5.2.3.1
1.5.2.3.1
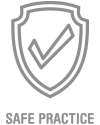
Gassing also releases hydrogen and oxygen, as we described in Lead-Acid Batteries in SECTION 1.2.2. If a flooded battery is installed inside a building, it’s important to provide an adequate air exchange to control the concentration of hydrogen well below explosive levels. Manufacturers’ application manuals provide formulas for calculating the amount of air required, based on battery type, size and age, float voltage or float current, and the number of cells. The manuals generally provide additional techniques for estimating the amount of water that will need to be replaced because of electrolysis in normal operation. More frequent watering may be required in applications with elevated temperatures.
One more thing: in a flooded cell with antimony alloy grids, gassing also releases stibine, a gaseous compound of antimony (antimony hydride). Stibine is poisonous, and in extreme cases can cause kidney failure. Ventilation adequate for hydrogen evolution also protects against stibine build-up.
Specific Gravity
1.5.2.4
Now consider specific gravity. What’s happening with that electrolyte during charging? In Discharging Secondary Batteries (SECTION 1.4), we pointed out that the specific gravity decreases during discharge, basically turning to water. In the manufacturer’s spec sheet, the specific gravity is usually given, but that’s for a new, fully-charged battery at room temperature, with Venus visible at sunset.
At the end of the discharge, the electrolyte is depleted, and is a poor conductor. During charging, the specific gravity increases, and the battery impedance decreases. As the specific gravity approaches its nominal value, it becomes more difficult to get in those last few ampere hours. While we talk about an eight-hour recharge, it may take a week or more on float charging to bring a stationary battery to full charge. Motive power batteries, because they’re usually charged by a two-rate constant current, may get closer to full charge in an eight-hour shift, but at the expense of more gassing.
Battery manufacturers recommend that the specific gravity of each cell be checked during periodic maintenance. These measurements, made with a hydrometer, should be performed by a trained technician. The readings must be corrected for temperature, since the nominal specific gravity is expressed at room temperature.
A note about NiCd and NiMH batteries: In these systems, the electrolyte (KOH) acts only as a medium for exchanging ions, and it’s the ion exchange that drives the external current. The specific gravity doesn’t change during discharge or subsequent recharge, and so the internal impedance is more constant than in a lead-acid battery. You can see this in the flatter discharge curve of voltage vs. time.
Electrolyte Stratification
1.5.2.5
Back to lead-acid batteries for a moment. In flooded cells, the electrolyte would ideally be evenly distributed throughout the cell. That is, the specific gravity would be the same, no matter where you measure it. But the electrolyte in a flooded cell has high mobility and, to boot, sulfuric acid is heavier than water. In a cell that has been on float charge for an extended period (or off charge completely), the higher density acid tends to sink to the bottom of the cell, leaving a weaker electrolyte at the top of the cell. Higher acid concentration at the bottoms of the plates can cause excessive sulfation of the grids.
During a discharge, the active material at the bottom of the cell provides more of the discharge current than the stuff at the top of the cell. The result is a plate that is unevenly discharged from top to bottom. On subsequent recharge, the tops of the plates will be overcharged before the bottoms of the plates are fully recharged, causing more grid corrosion and all the other nasty things that shorten battery life.
How to overcome this? Of course, you could pick up the cell and shake it, but I’m not applying for that job. Some large cells are equipped with stirring or aeration mechanisms to keep the electrolyte circulating. Equalize charging, and the gassing that results, is a good way to stir the electrolyte. Of course, this leads to increased maintenance.
By the way, the shaking method is more or less automatic in your car battery if you have a flooded design. If not, don’t worry: VRLA batteries are far less prone to stratification, since the electrolyte is absorbed or gelled, resulting in lower mobility.
Sulfation
1.5.2.6
When a lead-acid battery is discharged, the active materials are converted to lead sulfate, of a form that can be re-converted to the original active material during recharging in normal use. There is always a speck, though, that doesn’t get reconverted. This material eventually grows into a larger crystal structure, which resists charging. Over time, and many recharge cycles, the amount of crystal growth increases, resulting in grid growth; this robs the battery of useful active material, and therefore capacity. It also reduces the specific gravity over time.
Sulfation can be accelerated if a battery is left in a discharged state because the crystal growth is a slow but continuous process. Unlike NiCd cells, lead-acid cells cannot be stored in a discharged state, and in fact must be periodically refreshed when in storage.
Grid Corrosion
1.5.2.7
This occurs during the final phases of charging, when some of the charging current is going into electrolysis. The problem is chiefly at the positive plate, where oxygen is evolved; the oxygen reacts with the grid and active materials, causing the active material to lose contact with the grid, reducing capacity and increasing internal resistance.
In VRLA cells, the evolved oxygen and hydrogen are encouraged to recombine, replacing the water lost by electrolysis. However, there is always some corrosion, with attendant loss of electrolyte, and this is one of the limitations to the life of a VRLA cell.
Active Material Shedding
1.5.2.8
During discharging of a flat plate (flooded) cell, the plate enlarges because lead sulfate takes up a little more room than lead peroxide. Conversely, recharging causes the plate to contract again. This mechanical action gradually causes active material to be loosened from the plate, as pictured in Figure 1g. After it loosens, the material settles to the bottom of the cell jar.
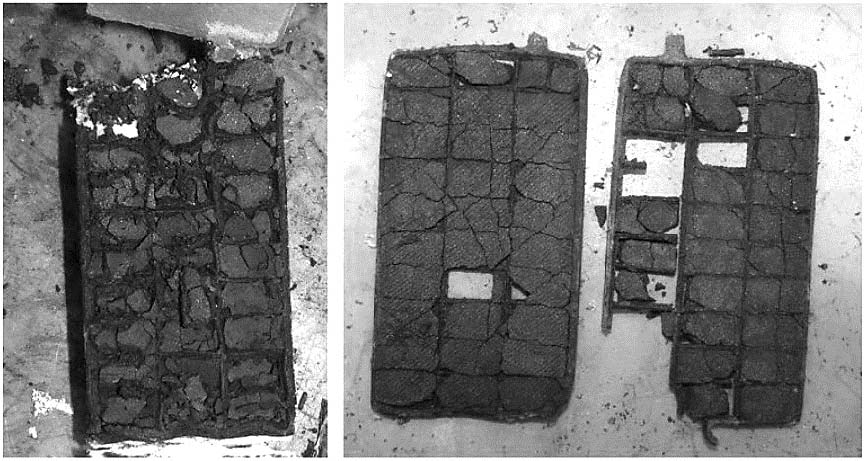
Flooded cells are constructed with a space under the plates for the shed material to collect. During the cell’s lifetime, the material gradually fills this space, and eventually may contact the plates above the space, shorting the battery. This prevents the cell from taking a charge, so that it must be serviced or replaced. At this point, the battery might have reached its end of life anyway, since serious shedding is one sign of frequent deep cycling.
FLOAT & EQUALIZE VOLTAGES: HOW DO THEY DIFFER?
1.5.3
Remember specific gravity? In lead-acid cells, the specific gravity determines the open-circuit voltage of the cell: the voltage is 0.85* plus the specific gravity. For example, a cell with a fully-charged specific gravity of 1.215 has an open-circuit voltage of 2.065 volts.
Why do batteries need to be recharged regularly, even if they aren’t used?
1.5.3.1
Like any electrochemical system, though, a lead-acid cell can’t just sit on a shelf and hold its charge forever. A lead-acid cell self-discharges through chemical action between the electrolyte and active materials, even though it’s delivering no external current. A cell in storage needs to have a refreshing charge at intervals no longer than about six months; if a cell is allowed to self-discharge for a longer period, some active material, and therefore cell Ah capacity, will be irretrievably lost.
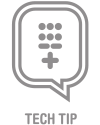
As the cell self-discharges, the specific gravity decreases, and the open-circuit voltage decreases along with it. So, we connect the cell to a charger and restore it to full charge. We know when we’re done because the specific gravity will be back at the proper value. If we can’t measure the specific gravity, we can go by the open-circuit voltage. This freshening charge is usually done at about 2.4 VPC.
What about just keeping a battery connected to a charger permanently?
1.5.3.2
Stationary batteries are normally permanently connected to a charger, which maintains the charge on the battery while also providing power to the connected dc loads. The charger is adjusted to a float voltage that just compensates for the self-discharge of the battery. The float voltage must be within the acceptable voltage range of all the equipment on the dc bus. [See also SECTION 1.4.5, How Do I Size A Stationary Battery?]
How do we know the proper float voltage?
1.5.3.3
In general, the manufacturer’s spec sheet provides this information. If this isn’t available, but you know the nominal specific gravity, you can calculate the open-circuit voltage. An acceptable float voltage would be 1.05 times the open-circuit voltage for lead-antimony, or about 1.08 times for lead-calcium.
Don’t know the specific gravity, either? You’re on thin ice, but you can try the following technique: Once you are sure the battery is fully charged, measure the float current while you adjust the float voltage. Try to get the float current within these ranges:
Lead-antimony 40 ± 20 mA per 100 Ah
Lead-calcium 11 ± 5 mA per 100 Ah
Lead-selenium 20 ± 10 mA per 100 Ah
These numbers are for room temperature, with the battery capacity given for the eight-hour rate. And, of course, the battery must be healthy, and nearly new, since float current rises gradually as the battery ages.
How long can stationary batteries remain on float charging without problems?
1.5.3.4
Stationary batteries can remain on float charging for a very long time without significant degradation and perform admirably at the next power emergency. There is a fly in the ointment, though (isn’t there always?). Maybe two flies. The first is that multiple cells connected in series, when on float for long periods, may become mismatched in capacity. This would be evidenced by differences in specific gravity (or open-circuit voltage) from cell to cell.
The second fly shows up when a battery is frequently cycled. Normally, you don’t want to do this with stationary batteries, especially calcium alloy, but it happens. With repeated discharge-charge cycles, some cells won’t be returned to full charge as soon as others; this may cause some cells to be overcharged more, causing more gassing.
What is equalization charge? How does it help prevent these problems?
1.5.3.5
The cure is the same in both cases: an equalization charge. This is a charge at voltage higher than float for a fixed period. The intent is to bring the “weaker” cells up to full charge so that they are matched with the stronger cells.
Note, though, that the terms “weaker” and “stronger” don’t refer directly to cell capacity or quality, but to the on-charge voltage for the cells.
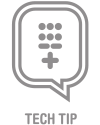
You’re saying, “But won’t that overcharge the stronger cells even more?” Well, yes. And it’s the reason that we normally equalize only flooded batteries, so that we can replenish the water that is inevitably consumed. VRLA batteries normally don’t need equalization, and most manufacturers recommend against it. It’s also important to terminate the equalize charge after a reasonable time; spec sheets usually recommend 12 to 24 hours. Also, equalize charging should be infrequent, preferably only when it’s indicated by imbalanced specific gravity or voltage readings. In some installations, equalize charging is initiated automatically after a power failure, when the ac power is restored. This automatic operation is predicated on the theory that the cells become mismatched during the previous discharge. For more on Equalization Charging, see SECTION 1.5.5.
Keeping the charger set to equalize will accomplish one thing: it will wear out the battery sooner.
CHARGE PROFILES: WHAT ARE COMMON METHODS FOR RECHARGING?
1.5.4
Goals
1.5.4.1
The goals of any charge profile are obvious: recharge the battery to full capacity as quickly as possible and make the battery last forever. Oh, and make sure you don’t overtax the charger components, or the dc bus wiring, or the ac power source, and keep the charging voltage (for stationary batteries) within spec for the connected loads. There are several approaches, depending on the battery application.
Motive Power: I-V-I Charge Profile
1.5.4.1.1
Motive power batteries are generally recharged with a constant current profile, at least for the start of charging. A popular profile is called the I-V-I (or I-U-I in Europe): a starting constant current that can be as high as C/2 (where C is the Ah rating of the battery), followed by a constant voltage period, and finishing with another constant current at a low rate.
The changeover from constant current to constant voltage is triggered by the battery reaching a critical voltage, usually 2.3 to 2.4 VPC, just below the onset of “hard” gassing. If the starting current is chosen correctly (about the C/2 to C/4 rate), this occurs at roughly 80% state of charge. During the constant voltage period, which may be set at about 2.4 VPC, the current gradually decreases until it reaches the finishing current, which can be set to C/10 to C/20. At that point, the charger reverts to constant current at the low rate and allows the voltage to rise without limit.
Normally, a timer terminates the charging. The timer may be started at the start of the charging cycle or be triggered by the switchover to the finishing rate. In the latter case, there should be an override timer, in case the current doesn’t decrease to the finish rate (and start the timer), which could be caused, for example, by a shorted cell.
A graph of this profile is shown in Figure 1h.
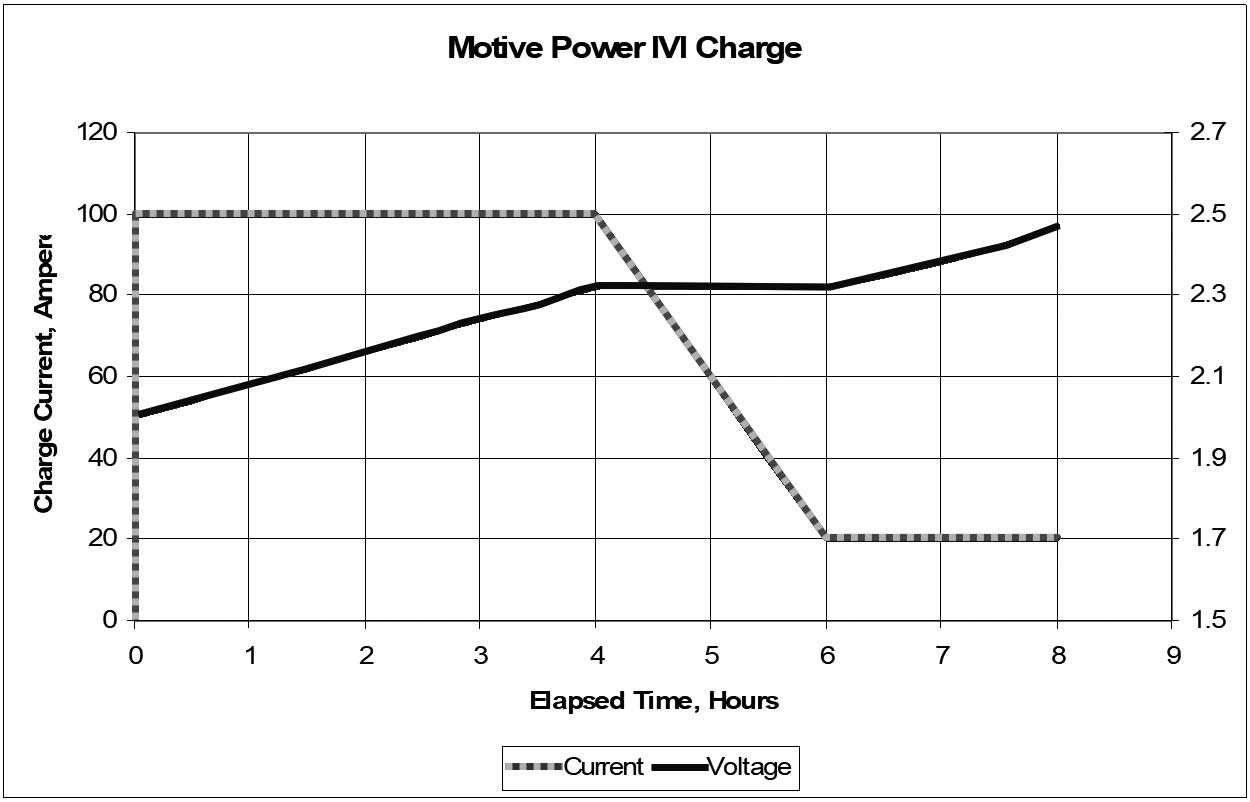
Stationary: Constant Voltage
1.5.4.1.2
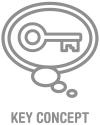
Stationary batteries are recharged at constant voltage. Both the float and equalize voltages are chosen to be within the acceptable range for the dc equipment connected to the battery. This means that specifying the float and equalize voltages is an integral part of sizing the battery, as is choosing the correct number of cells for the application (See How Do I Size a Stationary Battery, SECTION 1.4.5).
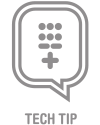
Normally, a charger is sized to recharge the battery in eight hours, while still powering the total connected load. This is fine in applications where the load is constant. In some applications, however, the load isn’t connected until there is an ac power failure, or the load is intermittent during normal operation. In a substation, for example, the charger is usually sized to recharge the battery without a dc load connected, or with very small load current.
Float chargers incorporate output current limiting (what’s that? See CHAPTER 4 Output Current Limit). When we first start to recharge a discharged battery, the charger is limited to a current that is usually 100% to 110% of the charger rating (not the battery rating). In a substation, for example, a charger might be rated to charge in eight hours, meaning that it would start at about the C/6 rate. Starting at the C/8 rate wouldn’t restore enough charge in eight hours because the charge acceptance for the last 10% or 20% isn’t as good as for the first 80%.
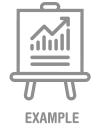
EQUALIZATION CHARGING
1.5.5
In SECTION 1.5.3.4 and SECTION 1.5.2.5, respectively, we described the need of a flooded lead-acid battery for occasional equalization – a recharge cycle at a voltage slightly higher than float voltage – to re-balance cell capacities and correct any electrolyte stratification.
What constraints must I consider when equalizing stationary batteries?
1.5.5.1
Voltage Limits on Connected Equipment
1.5.5.1.1
Electrical and electronic equipment powered by a dc bus has a power supply voltage “window” for safe and reliable operation. Equipment on a 24 Vdc bus may have an upper supply voltage limit of 30 Vdc; on a 125 Vdc bus, the upper limit is usually 150 Vdc.
A dc system design that includes equalization must keep these voltage limits in mind. The maximum equalize voltage for the battery must be less than the lowest upper-voltage limit for any connected equipment. This dictates the maximum number of cells allowed for the chosen cell type, based on the cell’s required equalization voltage. For more on this, see How Do I Size a Stationary Battery? in SECTION 1.4.5. If you’re using a temperature-compensated charger, you need to know the maximum voltage at the battery’s lowest operating temperature at your facility.
If you have a VRLA that doesn’t require equalization, it’s a good idea to disable the equalization capability of the charger or ensure that the equalize voltage is set to the same value as the float voltage.
In the previous section on charge profiles, we described float charging for stationary batteries. When a charging profile (sometimes called a charge regime) includes equalization, it is usually a two-voltage profile, with the higher equalize voltage being held for some time. The equalize time can be fixed, or be proportional to a battery charging characteristic, such as the time required for the on-charge voltage to reach a critical point, as in the profile for motive power batteries.
End Cells & CEMF Devices
1.5.5.1.2
Even with careful planning and cell selection, there may be applications where equalization is required, and the resulting dc voltage would exceed the load’s maximum specs. One solution is to disconnect the load while equalizing; this is rarely a viable option but may be possible in installations with redundant batteries.
A time-tested method to allow high voltage equalization is to use “Counter EMF,” or CEMF cells. Historically, one or two extra electrochemical cells were inserted between the battery and the load; the cells were usually unformed alkaline cells, meaning that the active material had nearly zero capacity. When the charger was set to the equalize mode, these cells provided a voltage drop between the battery and the load to hold the voltage on the load below the maximum allowed value. During float charging, or during discharge, the cells were shorted out with a contactor.
This method is like the "End Cell" concept, originally used in telephony, which inserted low-capacity cells in series during battery discharge to maintain usable bus voltage.
The obvious disadvantage to CEMF cells is that they increase maintenance requirements, since they use a liquid electrolyte which must be replenished. Electrolyte is lost during equalization charging because CEMF cells gas copiously. Modern CEMF devices use silicon diodes in place of electrochemical cells, and automatically-controlled switching devices to insert or remove the devices from the circuit.
How do I equalize if I have chargers connected in parallel?
1.5.5.2
If you have two (or more) chargers connected in parallel, you have a choice of two modes of operation: random load sharing and forced load sharing. More information on parallel charger operation is found in the first section of CHAPTER 7.
If you are operating two chargers with forced load sharing, both chargers must be set to the same operating mode – float or equalize – for the output load current to be shared. With load sharing enabled on some chargers, such as HindlePower’s AT series and ATevo charger, when you set the primary charger to equalize; the secondary charger is controlled by the primary, so that both remain in equalize mode until the end of the equalize charge.
One caveat with parallel chargers: There may be cases where a battery cannot safely accept the combined initial charge current of both chargers after a power failure. This might happen if the load on the dc bus is light when ac power is restored. In this case, you may have to reduce the current limit settings of both chargers.
What happens in a shorted cell?
1.5.5.3
Shorts happen. Through manufacturing defects, physical damage, or normal aging, a cell in a string may become shorted.* This reduces the back EMF of the battery by 2 Volts or so. In equalize charging, that 2 Volts must be absorbed by the remaining cells.
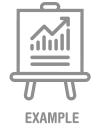
HOW DOES TEMPERATURE AFFECT BATTERY CHARGING?
1.5.6
We previously talked about temperature effects on batteries during discharge (SECTION 1.4.6). It’s not surprising that temperature excursions can also affect batteries during charging. Only it’s worse.
A lead-acid battery has a negative coefficient of on-charge voltage; as battery temperature increases, the terminal voltage required to maintain the same charge current decreases.
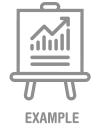
Why should we care? The most important answer is that if we don’t adjust the charging voltage at higher temperatures, we’ll use a lot more water, and generate more hydrogen and oxygen, that must be vented out of the battery room. If the battery is an antimony alloy, it’s possible to produce stibine, which, you remember, is poisonous. The gassing produces more grid corrosion. If it’s calcium alloy, we may alter the grid’s grain structure, reducing the amount of active material available for discharge. At higher temperatures, the self-discharge rate increases, and even keeping the float current the same may allow more sulfate buildup.
Basically, we’ll wear out the battery even faster. There is already a shorter life expectancy for a battery that spends a significant amount of time at elevated temperatures – a reduction of 50% for each 10 °C (18 °F) of elevated temperature.
On the cool side, of course, we would have to raise the float voltage to maintain the same current, and a temperature-compensated charger will do just that. Without compensation, the battery might not reach full charge at low temperatures.
See more on Temperature Effects in CHAPTER 5.
DOES A “MAINTENANCE FREE” BATTERY NEED MAINTENANCE?
1.5.7
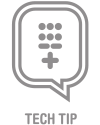
A battery is like your car: maximizing its useful service life requires routine maintenance. Even batteries advertised as “maintenance free” aren’t. Yes, maintenance is reduced because you don’t have to, or can’t, add water to the electrolyte, and you can’t measure the specific gravity. But you still need to keep the batteries clean, measure cell voltages on a regular basis, keep the intercell connections tight, and check for container cracks or potential leaks. If you find a leak, the offending unit must be replaced. Clean up any spills with sodium bicarbonate (watch for excess foaming); ammonia can also be used. Remember that lead-acid batteries are treated as hazardous materials, as is the electrolyte. Lead-acid batteries should be recycled (in fact, that’s the law in most states).
The most important maintenance task that you need to perform for a flooded battery is to maintain the proper level of electrolyte. Here’s how.
When and how do I add water?
1.5.7.1
Battery jars for flooded cells are transparent or translucent.* At the top of each cell section are two index marks, indicating the maximum and minimum electrolyte levels. The minimum level, as you would expect, is a comfortable distance above the tops of the plates.
Keep the electrolyte level roughly centered between the two marks. During discharge, as sulfuric acid combines with the active material of the plates, the level decreases because some of the sulfuric acid is combining with the active material in the plates. Likewise, the plates expand because lead sulfate takes up more room than the original active materials.
It’s tempting to add water at the end of the discharge, especially if the level has fallen below the lower index. Don’t. When you recharge the battery, the sulfuric acid returns to the electrolyte solution, and the electrolyte level in the cell rises above the index mark again.
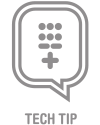
The time to add water is at or near the end of a complete recharge. If the level is not midway between the index marks, add enough water to bring it to that level. If you’re doing an equalize charge, you can add water during the last hour or so of the recharge, so that the gassing action during equalization will mix the electrolyte, avoiding stratification.
If a battery hasn’t been maintained properly for a while, the tops of the plates may be uncovered. In this case, add enough water to cover the tops of the plates, and do a complete recharge. Then add water, as before, to get to the right level.
If I’m only adding enough water to get to the midpoint, why is there even an upper index mark?
The smart aleck answer is so that you’ll know where the midpoint is. The real answer is so that you’ll know if too much water has been added or added too soon in a charge cycle.
Remember that the electrolyte level and specific gravity are related. When the battery is fully charged, and the level is correct, specific gravity will be at the manufacturer’s specified value. If the water level is too high in a cell (that is, above the upper index mark), the gravity will be low, even if the cell is fully charged. This means that the cell voltage will be low. If the electrolyte level is high enough to leak through the vent caps, it’s a good idea to remove some of the electrolyte. You will have to monitor and adjust the specific gravity over the next couple of charge cycles. If specific gravity is too low, battery capacity will be reduced. If it’s too high, corrosion will be increased.
Does the amount of water required depend on battery design?
1.5.7.2
Water usage varies widely among the various alloys and grid designs used for lead-acid cells. The oldest grid alloy in use, a so-called lead high-antimony alloy, has the lowest potential for electrolysis (meaning it starts to gas at a lower voltage). It also has a relatively high self-discharge rate. For this reason, this alloy is used only for flooded cells, and in most cases only for pasted-plate cells. The antimony alloy is superior for cycling applications, such as motive power batteries.
To reduce the maintenance requirements of cells, manufacturers have developed several other alloys. Lead-calcium alloys have been in use since the 1930s. They have the opposite set of characteristics from antimony alloys: much lower float current and water usage, low self-discharge rate, but relatively poor cycling ability. They are ideal for standby and stationary applications and are the alloy of choice for VRLA batteries.
Low-antimony alloys, often containing selenium, have lower water usage than high-antimony alloys, while maintaining good cycling ability.
Lead-tin alloys, sometimes marketed as “pure lead” batteries, can be used for VRLA batteries in high-rate applications. You probably won’t see this alloy in a flooded construction.